Implantable Microphone
We are developing a prototype implantable microphone as a component of a totally implantable cochlear implant (TICI). Unlike a regular cochlear implant, a TICI is invisible and can be used during sports and sleeping. Furthermore, the internal microphone will leverage the pressure gain and directional cues provided by the external ear, resulting in improved hearing. We plan to develop our implantable microphone to readiness for FDA approval testing. The prototype presented here is the third design we have developed, and is called the UmboMic or the IMic.
Background
Cochlear implants are a revolutionary invention that can restore hearing loss to people with damaged inner ears. However, they rely on an external component that holds the battery, sound processor, and microphone. This external part limits patient activity and is visually indiscreet. Furthermore, the external nature of the microphone means the device is susceptible to noise from wind and does not work well in loud environments. Implanting the external component will mitigate these issues and greatly improve the user experience. However, the most difficult part of developing a TICI is creating the microphone. We present the iMic as an approach to an implantable microphone.
Our approach
The iMic is a piezoelectric displacement sensor that detects the motion of the umbo, which is the tip of the malleus that attaches to the underside of the eardrum. When sound waves enter the ear canal and hit the eardrum, they cause the membrane to vibrate, which in turn moves the umbo. The umbo displaces the piezoelectric iMic, resulting in a measurable charge accumulation. The umbo’s motion is large and unidirectional, making it a better place to target than other parts of the ossicular chain.

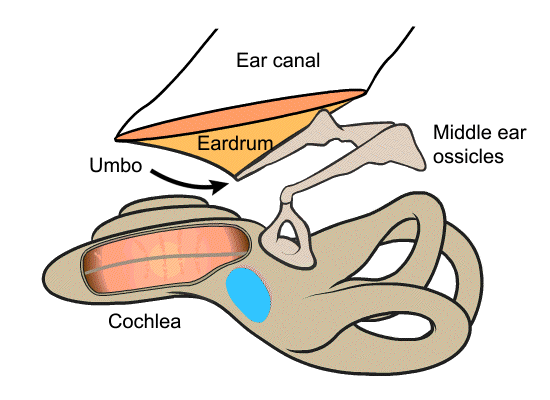
The iMic is shaped like a triangular diving board and is made from two layers of the piezoelectric thin-film, polyvinylidene fluoride (PVDF). This type of cantilever shaped structure with two active layers is known as a bimorph. The output from our sensor is the difference in charge between the top and bottom layer when the sensor is bent. We amplify this signal with our custom low-noise differential charge amplifier. A differential measurement is advantageous over a single ended measurement because it reduces common mode noise and thus boosts the signal to noise ratio. One of our previous designs used only a single layer of PVDF and it suffered from high noise and electromagnetic interference.
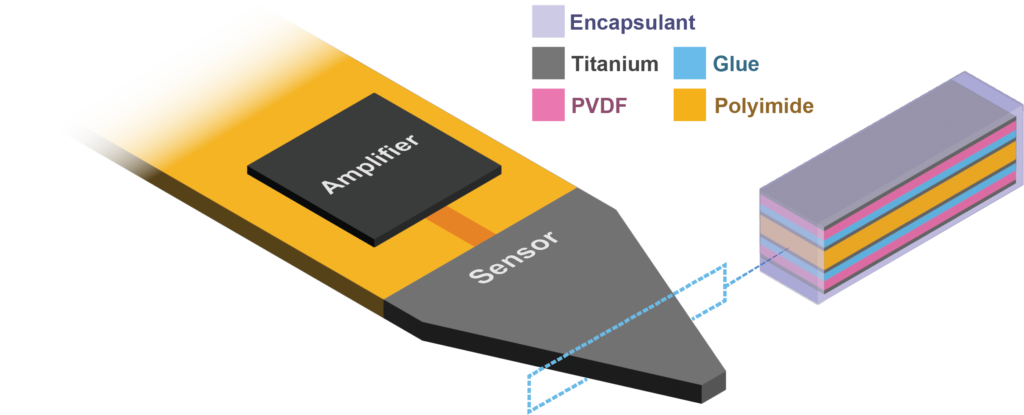
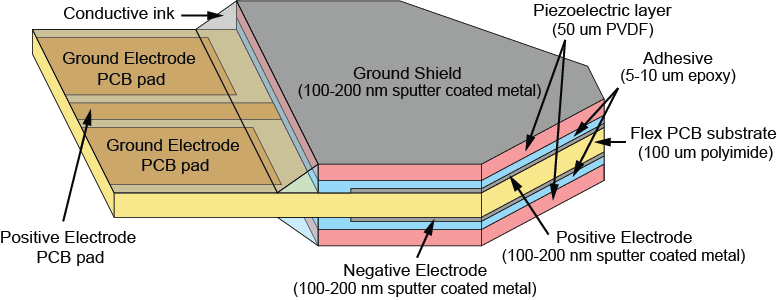
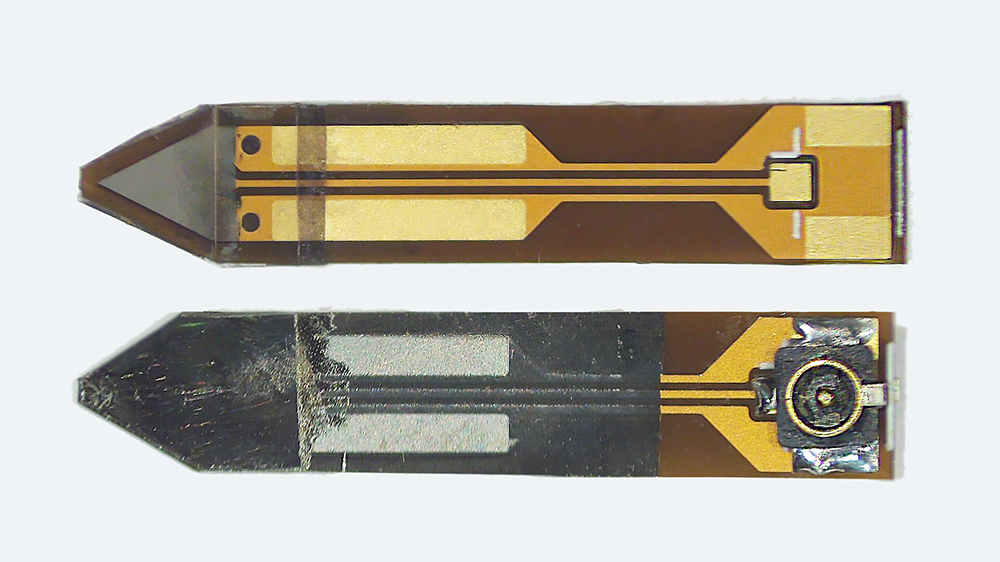
Testing and results
We test all of our iMic sensors in fresh cadaveric specimens provided by Mass Eye and Ear. The specimens are prepared in a manner similar to the surgery used for cochlear electrode insertion. This surgery allows access to the middle ear cavity, through which we can insert our sensor to contact the umbo.
We’ve demonstrated through extensive testing that the iMic has high sensitivity and a bandwidth up to at least 7 kHz. Its equivalent input noise is very low, comparable to that of commercially available hearing aids.
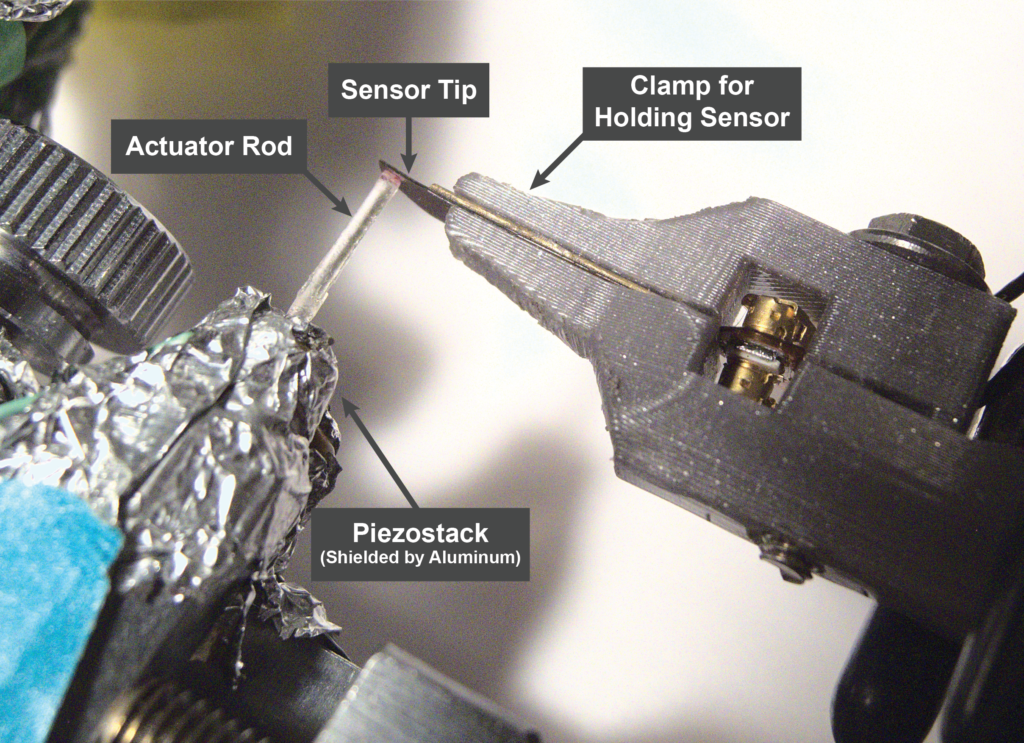
Current and future work
Our team is preparing to implant the iMic in live sheep as an animal model. Concurrently, we are working towards making the iMic biocompatible and robust to years of wear in the body. Choosing a robust and flexible encapsulation layer is key to the longevity of the implanted device, and we are studying various methods of encapsulation using accelerated aging.
Additionally, our team is iterating on a stable fixation device for holding the sensor inside of the middle ear canal. The related figure here shows a current locking ball joint design printed in titanium. Finally, we are considering redesign of the amplifier such that it is smaller and low power.
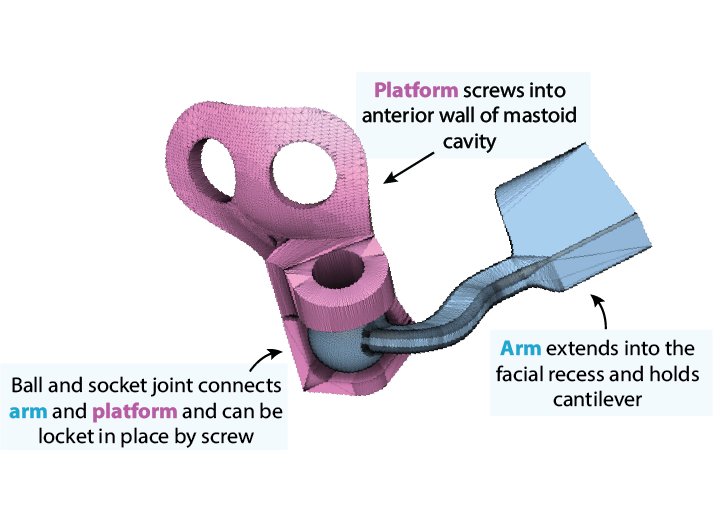
Who we are
Our team consists of a variety of research groups across different schools and disciplines.
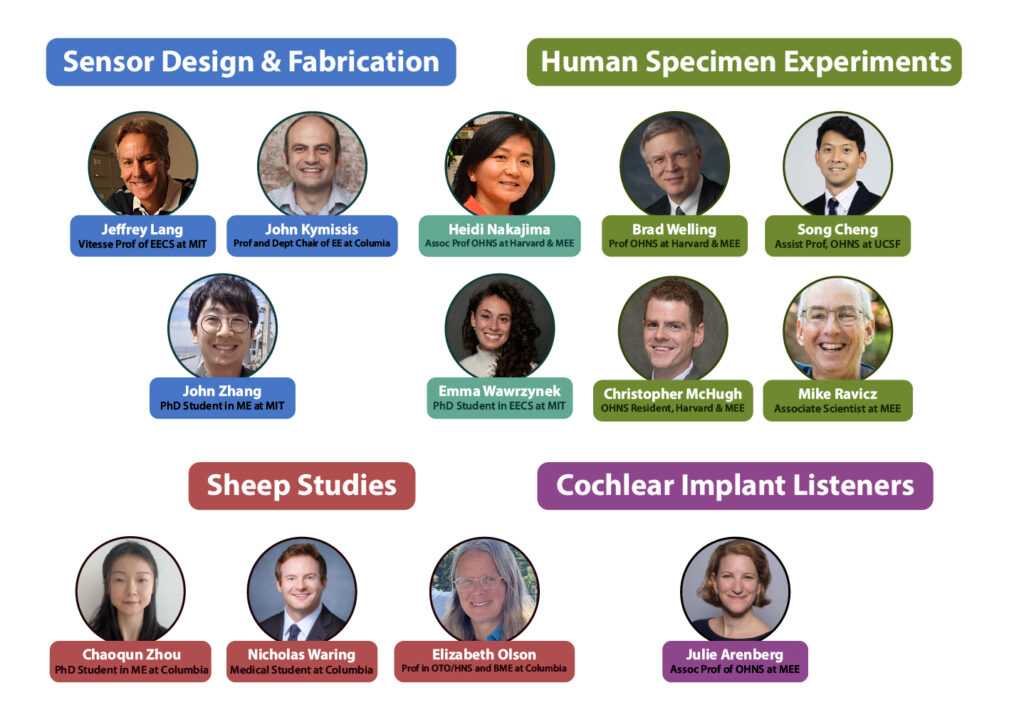
Human Cochlear Partition
The Nakajima lab studies human cochlear anatomy and function and how it contrasts to that in commonly studied animals. In particular, the lab focuses on the cochlear partition, composed of the osseous spiral lamina, basilar membrane, and the organ of Corti which is the organ responsible for translating mechanical motion into electrical signals that are sent to the brain. By studying the anatomy, measuring motion within the cochlea, and modeling the cochlear partition, we can get a fuller picture of the importance of cochlear anatomy as it relates to hearing in human.
Anatomy
There are some key differences between human cochlear anatomy and animal anatomy. For one, the osseous spiral lamina in the human is comparatively longer and flatter than the short tapered osseous spiral lamina in commonly studied lab animals. Another difference is that the human cochlea contains a soft tissue bridge region between the osseous spiral lamina and the basilar membrane. Consequently, components of the organ of Corti are positioned differently on the basilar membrane and may translate to differences in mechanical transduction. By studying anatomy via histology and optical coherence tomography imaging, Paul Secchia is quantifying the anatomical differences in human temporal bones.

Vibrometry
This difference in human anatomy relates to its difference in function: the osseous spiral lamina is able to move like a diving board, compared to in animal where it is relatively immobile. In collaboration with Sunil Puria’s lab, Paul Secchia is using optical coherence tomography to measure submicron motion of the cochlear partition structures.
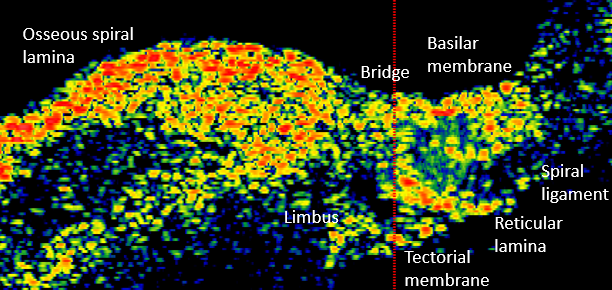
Modeling
Finite element modeling of the human cochlear partition can be used to supplement anatomical and vibration measurements to help make sense of them. Using experimental data as calibration, Andrew Tubelli builds a finite element model of the human cochlear partition and then varies the mechanical properties of the partition stuctures to better understand how they relate to function.
Past Research Topics
Temporal Bone Research
Bone Conduction
The ears are the primary way to perceive sound, but the skull provides another pathway for sound to be interpreted by the sensory organ in the ear. Bone conduction has been used as a diagnostic tool and treatment for hearing loss since the 17th century. Recently there has been a growing popularity of bone conduction devices which allow for audio communication without blocking sound entering through the ear canal. With the innovative miniature pressure sensors, we are able to study the mechanisms of bone conduction. We also have an interest in understanding effects of diseases, such as otosclerosis and semicircular canal dehiscence, on bone conduction hearing.
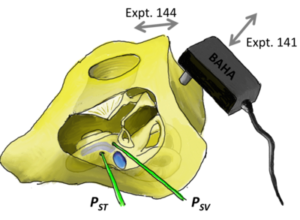
Round Window Stimulation Device
Instead of introducing sound to the inner ear by mechanically stimulating the oval window (as in normal air conduction – AC), the cochlea can be driven in “reverse” by mechanically stimulating the round window (RW), which is a pressure release system of the cochlea. The Nakajima lab is creating a device that helps those with outer and/or middle ear problems by stimulating the RW membrane. We focus on increasing the coupling of the device to any RW membrane despite variations in ear anatomy. We are currently testing new designs and have a patent filed.
Modeling the Middle and Inner Ear
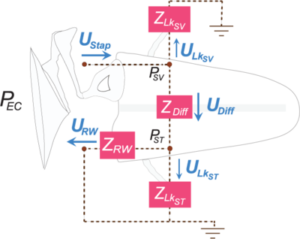
Our lab specializes in intracochlear pressure sensor measurements and we’ve accumulated enough data from many ears to create an impedance model of the middle and inner ear. Analyses of intracochlear pressures in the scala vestibuli (SV) and scala tympani (ST), as well as velocities of the middle ear, enables us to quantify the frequency-dependent impedances in the middle ear and cochlear system. Our analyses also further the understanding of air conduction (AC, pictured) and RW stimulation by quantifying the volume velocities of various paths of sound during different stimulation modes.
Low-frequency hearing and infrasound propagation through the middle and inner ear
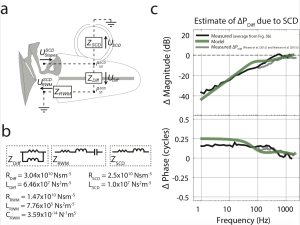
It is widely believed that the hearing range of humans is between 20 Hz to 20 kHz (as described above). However, there is compelling evidence that infrasound (frequencies below 20 Hz) does enter the ear and even alter sound processing in the cochlea. Especially in industrialized nations, people are frequently exposed to high-energy, low-frequency sounds radiated by heavy machinery, making exposure to infrasound a possible public health concern. Yet the transmission of infrasound to and within the inner ear is not well described.
We measured the motion of middle ear bones and the round window membrane (part of the inner ear) for frequencies between 1 Hz and 2000 Hz and show that the middle ear considerably limits the sound energy transmitted to the inner ear at such low frequencies. However, inner ear pathologies such as semicircular canal dehiscence (SCD) decreases the velocity of the round window membrane in a predictable, frequency-dependent manner, demonstrating that acoustic energy is shunted through and exciting the vestibular system. Our findings suggest that mechanical perturbations of the inner ear could lead to vestibular hypersensitivity to infrasound.
Clinical Research
Superior Canal Dehiscence
First described by Minor and colleagues in 1998, superior canal dehiscence (SCD) is a disorder characterized by a defect in the bony covering of the superior semicircular canal in the inner ear. Patients with SCD experience a range of auditory and/or vestibular complaints. Although believed to be rare, knowledge and awareness of SCD among physicians has increased over the past decade. However, the diagnosis is difficult to establish as the presentation of SCD often mimics symptoms of other common ear pathologies. As a consequence, patients may wait years before getting their diagnosis and have often receive incorrect treatment for other pathologies.
Our lab is interested in understanding the underlying mechanics of the inner and middle ear in patients with SCD and to develop methods to establish the diagnosis of SCD in patients. We perform wideband acoustic immittance and laser Doppler vibrometry in patients diagnosed with SCD at our institution. Furthermore, we compare measurements before and after surgery to assess the mechanical effect of surgery on SCD. In our lab, we model the SCD in fresh human temporal bone specimens. The basic science work seeks to improve our understanding of the mechanisms behind SCD and to quantitatively study the effects of different surgical approaches to improve SCD treatment.
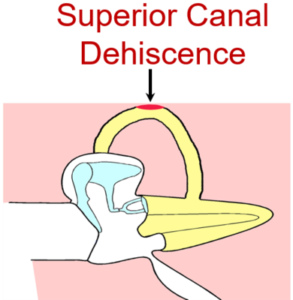
Development of computational models to diagnose mechanical lesions of the ear
Current clinical test reveal the cause of conductive hearing loss (CHL) in majority of patients, but there is a subset of patients with CHL in the aerated middle ear and intact tympanic membrane who present a diagnostic dilemma. The differential diagnosis in this setting include ossicular fixation or discontinuity, and third window disorders such as superior canal dehiscence. We have demonstrated the potential of Ear Canal Reflectance (ECR) and Laser-Doppler vibrometry (LDV) measurements in this subset of patients as a non-invasive diagnostic tool, which is valuable for patient counselling, surgical planning and may even spare patients from unneccesary diagnostic middle ear exploratory surgery. This project seeks to evaluate non-invasive diagnostic measurements such as wideband acoustic immittance and laser Doppler vibrometry for their efficacy in 1) detecting various macro-mechanical pathologies of the ear with computational models, and 2) monitoring the mechanical changes of the ear following surgical repair.
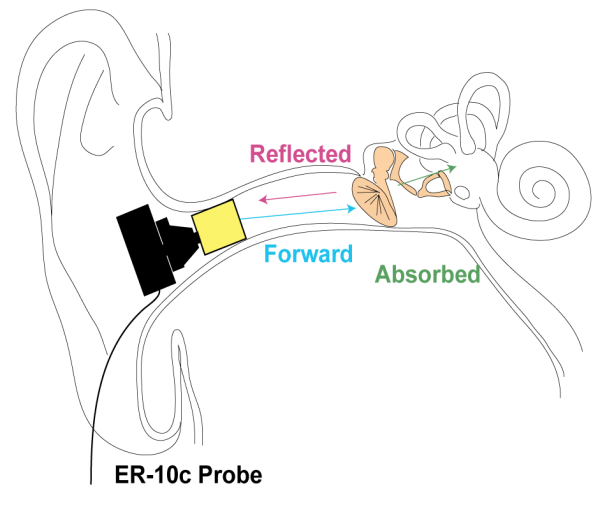
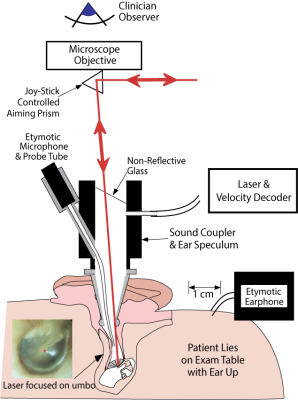